1. Introduction
The role of mitochondria, at the crossroads of many studies related to cancer invasiveness, has been extensively investigated over the last fifteen years [
1]. Their involvement in motility and invasion, microenvironment, plasticity, and colonization was recently reviewed [
2]. Since the pioneering work of Ishikawa et al. demonstrating the role of mtDNA transfer in the acquisition of high metastatic potential [
3], cancer cells were shown to acquire mitochondria from neighboring cells to acquire phenotypic characteristics, including stemness, representing gain of functions for tumors, enhancing their invasive properties [
4]. The interplay between mitochondrial dynamics and extracellular matrix (ECM) remodeling was emphasized [
5], and the molecular mechanisms linking dysregulated fission / fusion to tumor progression and metastasis deciphered [
6]. Together with an update view of mitochondria dysfunction on tumor glycolysis [
7], these important breakthroughs have a profound impact on new therapeutic strategies aiming at overcoming hypoxic and chemorefractory tumors [
8]. Finally, the upregulation of mitochondrial proteins involved both in ATP production and drug resistance [
9], and/or immune-resistance [
10] could lead to new therapies. In parallel, all these studies could also shed light on new biomarkers for better predicting cancer chemosensitivity [
11].
In this context, proteomics-based investigations provide crucial insights into the role of mitochondrial proteome rewiring [
12,
13,
14]. The development of high-throughput proteomics techniques, combined with the use of experimental models of increasing invasiveness, has led to the identification of new proteins of interest both in rats and humans [
15]. Given this methodological background, in this study we aim to focus on mitochondrial proteins, which appear to play an important role in metabolic rewiring and the invasiveness process both in experimental tumor models and tumor cells from patients.
2. Materials and Methods
2.1. Collection of rat tumor tissues for proteomic analyses
The formalin-fixed paraffin embedded (FFPE) tissue samples used in this study were collected from the same groups of Fisher F344 rats with four different experimental mesotheliomas at increasing stages of invasiveness, as previously described [
16]. To generate the tumors, the experimental procedures used for in vivo manipulations at the UTE-IRS UN (Unité Thérapeutique Expérimentale de l’Institut de Recherche en Santé de l’Université de Nantes) between 2011 and 2015 followed the European Union guidelines for the care and use of laboratory animals in research protocols (approval #01257.03 from the French Ministry of Higher Education and Research (MESR)). The rats were purchased from Charles River Laboratories (L’Arbresle, 69210, France) and the experiments were approved by the ethics committee for animal experiments (CEEA) of the Pays de la Loire Region registered under the number 2011.38. The non-invasive M5-T2, mildly invasive F4-T2, moderately invasive F5-T1 and deeply invasive M5-T1 tumors were collected after intraperitoneal injection of 3 x 106 cells of the corresponding cell lines (
https://technology-offers.inserm-transfert.com/offer/, recorded as RT00418, RT00419, RT00421 and RT00417, respectively) into syngeneic rats.
2.2. Proteomic analyses
For each sample analyzed, four-five 20-µm-thick sections of tumor tissue were scratched with a scalpel and collected in a 1.5-mL Eppendorf® microtube, and all the material collected was deparaffinized in three successive xylene washes, then rehydrated in 100%, 95%, 70% and 50% ethanol solutions. The pellets were vacuum-dried, and the dried tissues resuspended in 200 µL of Rapigest SF (Waters, Milford, MA, USA). Dithiothreitol (AppliChem, Darmstadt, Germany) was then added (5 mM final concentration), and the samples incubated in a thermo shaker at 95°C for one hour, and then sonicated twice (ultrasonic processor 75,185, Bioblock Scientific, Illkirch, 67400, France). Cystein residues were alkylated by adding 200 mM S-Methyl methanethiosulfonate at 37°C (10 mM final concentration). Sequencing-grade trypsin was added at a ratio ≥ 2 µg mm-3 tissue (at 37°C overnight), the reaction was stopped with formic acid (9% final concentration, incubation at 37°C for one hour), and the acid-treated samples were centrifuged at 16,000 g for 10 minutes. After removing the salts from the supernatant, the peptides were collected in a new Eppendorf® microtube using C18 STAGE tips, and their concentration finally determined using the Micro BCA™ Protein Assay Kit (Thermo Fisher Scientific, St Herblain, 44800, France). The rat spectral library, SWATH-MS analysis, peptide identification, and relative quantification were performed as previously described [
16]. The statistical analysis of the SWATH data set and peak extraction output data matrix from PeakView was imported into MarkerView (v.2, AB Sciex Pte, Ltd., Framingham, MA, USA) for data normalization, and relative protein quantification. Proteins with a statistical
p-value < 0.05 estimated by MarkerView were considered differentially expressed under different conditions.
2.3. Histology and immunohistochemical analyses
The FFPE blocs were cut with a Leica RM2255 microtome (Leica Biosystems, Nussloch, Germany). Areas of interest for both proteomic and histological analyses were selected based on examination of sections of all samples stained with hematoxylin phloxine saffron (HPS), scanned on a Nanozoomer 2.0 HT Hamamatsu. For immunohistochemistry, tumor sections were stained with anti-ACADL NBP2-92854 polyclonal antibody (Novus Biologicals, Centennial, CO 80112, USA).
2.4. Chemicals
FBS and culture medium were from Invitrogen Life Technologies. Plasticware for cell cultures was from Falcon (Becton Dickinson). The protein content was assessed with the BCA Kit from Sigma Chemical Co. Electrophoresis reagents were obtained from Bio-Rad Laboratories. Unless otherwise specified, all the other reagents were purchased from Sigma Chemical Co.
2.5. Cells
Ten primary human MM cell lines (5 epithelioid and 5 sarcomatoid), obtained during diagnostic thoracoscopies, were collected from the Biologic Bank of Malignant Mesothelioma, S. Antonio e Biagio e Cesare Arrigo Hospital (Alessandria, Italy), after written informed consent. The local Ethics Committees approved the study (#9/11/2011; #126/2016). Cultures were used within passage 10. Clinical and pathological characteristics of the MPM patients are reported in
Table 1. Primary MPM cells were grown in HAM’s F12 and DMEM medium, supplemented with 10% v/v fetal bovine serum and 100 U/ml penicillin-100 μg/ml streptomycin.
2.6. Immunoblotting
Cells were rinsed with lysis buffer (150 mM NaCl; 1.0% Nonidet P-40; 50 mM Tris-Cl; pH 7.4), supplemented with the protease inhibitor cocktail, sonicated and centrifuged (13,000 ×g, for 10 min at 4°C). 20 μg of proteins were probed with the following antibodies: ACADL (ab152160, Abcam, Cambridge, UK); β-tubulin (sc-52-74, Santa Cruz Biotechnology Inc.), followed by peroxidase-conjugated secondary antibodies (Bio-Rad Laboratories, Hercules, CA). Blots were washed with Tris-buffered saline/Tween 0.01% v/v, developed with enhanced chemiluminescence (Bio-Rad Laboratories) and imaged using a ChemiDocTM Touch Imaging System device (Bio-Rad Laboratories).
2.7. Mitochondria isolation
To isolate mitochondria, cells were washed twice in 8 mL of PBS 1X, then 0.8 mL of mitochondria lysis buffer (50 mM TRIS, 100 mM KCl, 5 mM MgCl2, 1 mM EDTA and 1.8 mM ATP, pH 7.2) mixed with protease inhibitor cocktail set III (100 µL). PMSF (100 µL) and NaF (25 µL) were added to lyse the cells. Cells were scraped and collected in an Eppendorf® tube, then sonicated twice for 10 seconds at 40% power. Subsequently, samples were centrifuged at 2000 rpm for 1 minute at 4°C. The supernatant was collected into a new series of Eppendorf® tubes and centrifuged again at 13000 rpm for 5 minutes at 4°C. Pellets containing mitochondria were washed with 0.4 mL of mitochondria lysis buffer and centrifuged at 13000 rpm for 5 minutes at 4°C. Subsequently, the supernatant was aspirated and the pellets resuspended in 0.2 mL of mitochondria resuspension buffer (Sucrose 250 mM, K2HPO4 15 mM, MgCl2 2 mM and EDTA 0.5 mM, pH 7.2). The resuspended mitochondria were divided: one part was used to measure mitochondria protein content using a BCA kit (Sigma). The remaining part of resuspended mitochondria was divided into 50 µL aliquots and stored at -80°C until use.
2.8. ETC (Electron transport chain from complex I to complex III)
The electron transport between complexes I and III was measured in mitochondrial extracts obtained previously. In particular, 10 µL of mitochondria samples were put in a 96-well plate together with 160 µL of buffer A (5 mM KH2PO4, 5 mM of MgCl2, 5% w/v bovine serum albumin, pH 7.2), 100 µL of buffer B (50 mM KH2PO4, 5 mM MgCl2, 5% w/v serum bovine albumin, 0.05% saponin, pH 7.5) and freshly added 0.12 mM of cytochrome c-oxidized form and 0.2 mM of NaN3. After waiting 5 minutes to equilibrate the plate at room temperature, 30 µL of NADH (0.15 mM and diluted in buffer B) were added to each well. The reaction then started, and the absorbance was read at 550 nm for 6 minutes, with 1 read every 15 seconds. Considering only the linear part of the curve and calculating results in accordance with Lambert-Beer equations, the results obtained were expressed as nmoles of cytochrome C reduced/min/mg mitochondrial protein.
2.9. ATP
ATP quantities were measured following the Sigma-Aldrich protocol 213-579-1. First 50 µL of ATP assay mix (lyophilized powder containing luciferase, luciferin, MgSO4, DTT, EDTA, BSA, and tricine buffer salts, pH 7.8) were added to a vial for 3 minutes. Then, 50 µL of sample (mitochondria extract obtained as described in the previous steps) were rapidly added and the quantity of light was measured in a black 96-well plate in a microplate reader. The results were expressed as nmols of ATP/mg mitochondrial.
2.10. β-oxidation of fatty acid
Assays were performed using the fatty acid complete oxidation kit (ab222944; Abcam, Cambridge, UK) as per the manufacturer’s instructions. Cells were plated at 40,000 cells per well in a 96-well plate with 200 µl of medium per well and left overnight to equilibrate. The cells were washed twice with prewarmed FA-free measurement media, incubated with FA measurement media (150 µM FAO-Conjugate; 0.5 mM L-Carnitine) with extracellular O2 consumption reagent (ab197243; Abcam, Cambridge, UK), and then sealed with mineral oil. The fluorescence signal was read in a microplate reader (Ex/Em = 380/650 nM). The results were expressed as pmoles of O2/min.
2.11. Scratch assay
Cells were plated at 1x106 cells per well in a 6-well plate. After 24h, scratches using a 20-200µL pipette tip were made. The cell migration was calculated measuring distance (in µM) between the cells at T0 (immediately after the scratch) and T1 (24h after the scratch) and dividing it for 24h. The results were expressed as µM/h.
2.12. Real time PCR (RT-PCR)
Total RNA was extracted using VWR Life Science RiboZol™ RNA Extraction Reagent (VWR Life Science) and reverse-transcribed using the iScriptTM cDNA Synthesis Kit (Bio-Rad Laboratories). qRT-PCR was carried out using SYBR Green Supermix (Bio-Rad Laboratories). qPrimerDepot software (
http://primerdepot.nci.nih.gov/) was used to obtain the following PCR primers (
Supplementary Table S1). Gene Expression Quantitation software (Bio-Rad Laboratories) was used to assess relative gene expression levels.
2.13. Statistical analysis
All data in the text and figures are provided as a mean ± SEM. The results were analyzed using a one-way ANOVA and Tukey test. P < 0.05 was considered significant.
4. Discussion
Cross-species investigations have provided new insights into universal mechanisms in biology, improving for example our understanding of oncogenic signatures in breast cancer development in humans and dogs [
31]. Applied to proteomic analyses in cancer, common biomarkers of invasiveness have been identified in rat and human mesotheliomas [
15]. Genomic analyses also pointed to reliable markers useful for the diagnosis and prognosis of hepatocellular carcinomas in both species [
32]. To date, cross-species comparisons of important findings relevant to mitochondria have been very limited, focusing for example on detecting heteroplasmy [
33]. In this study, we identified several biomarkers of interest that appear to play an important role in metabolic rewiring and invasiveness in both human and rat mesotheliomas.
As biosynthetic hubs, mitochondria consume a variety of different fuels to generate energy in the form of ATP for cancer cells, where fatty acid oxidation plays an important role [
34]. Although most cancer researchers initially focused on glycolysis, glutaminolysis and fatty acid synthesis, the relevance of fatty acid oxidation in the metabolic reprogramming of cancer cells was extensively reviewed 10 years ago, emphasizing its role in NADPH production [
35]. Linked to this statement, our results revealed two consistent findings regarding the FAO enzyme
ACADL, observed both in humans and rats, and associated with the acquisition of invasive properties. The higher expression of
ACADL was initially found to be positively correlated to prostate cancer progression [
36].
Our data also agreed with the work of Yu et al. showing that
ACADL was overexpressed both in cell lines and clinical specimens, being related to esophageal squamous cell carcinoma progression and poor diagnosis [
37]. Another close FAO enzyme, which is encoded by
HSD17B10, is also involved in branched amino acid catabolism and steroid metabolism. Our data are in line with previous published reports emphasizing its upregulation in invasive tumors. For example, Salas et al. have shown its predictive value in the response to chemotherapy in osteosarcomas [
38]. Its overexpression also accelerated cell growth, enhanced cell respiration, and increased cellular resistance to cell death in pheochromocytoma [
39]. Finally, and even more interestingly, Condon et al. found
HSD17B10 was one of the six genes impacting the mTORC1 pathway [
40], which is dysregulated and activated in cancer cells to drive survival, neovascularization, and invasion [
41].
Interestingly, the increased β-oxidation rate, electron flux and ATP production observed in human sarcomatoid mesothelioma cell lines were all consistent with the increased expression of ATP synthase subunits, cytochrome
c oxidase subunits, abundance changes in these proteins in rat tumors, and with our observations above on the long-chain acyl coenzyme A dehydrogenase. Fiorillo et al. have highlighted the fact that ATP-high cancer cells were phenotypically the most aggressive, with enhanced stem-like properties, multi-drug resistance potential and an increased capacity for cell migration, invasion, and metastasis [
42]. Wang et al. also pointed out that its high expression was linked to poor prognosis in glioblastoma, clear cell renal cell carcinoma, and ovarian, prostate, and breast cancers [
43]. Moreover, an additional role of ATP synthase in the formation of the permeability transition pore (PTP) was also recently reported as representing a mechanism controlling tumor cell death [
44]. In this process, our findings also tend to confirm the important role of the subunit
d of ATP synthase (encoded by (
Atp5h /
ATP5H), linked to the work by Chang et al. who reported the involvement of the overexpression of this subunit in venous invasion, distant metastasis of colon cancer, and finally poor survival [
45].
Within the enzymes of mitochondrial metabolism involved in cancer progression, besides isocitrate dehydrogenase and malate dehydrogenase, subunits of the cytochrome
c oxidase (complex IV of the respiratory chain), such as COX5B, were also reported [
46]. Our results agreed with previous published literature on the impact of its high expression on tumor invasiveness and poor prognosis in patients with breast cancer [
47]. More recently, further insights have confirmed its tremendous role as a growth-promoting gene both in hepatoma [
48] and colorectal cancer [
49]. Interestingly, the combined upregulation of COX5B and ATP5H was also reported by Yusenko et al. in renal oncocytomas [
20]. Another subunit of the cytochrome
c oxidase, COX6C, also upregulated in relation to invasiveness in our study, appeared to be differentially expressed in various cancers [
50]. An important finding by Jang et al. showed it was detected in extracellular vesicles (EV) in the plasma of metastatic melanoma, ovarian and breast cancer patients, suggesting the classic EV production and mitochondrial pathways were interconnected [
51]. In this latter study, an additional crucial observation was the presence of another inner mitochondrial membrane protein in these EVs [
51], encoded by
MTCO2. These breakthroughs are consistent with both the increased abundance of SODM and expression of this gene that we found in the most invasive rat tumors as well as in human mesothelioma cell lines. Linked to the tremendous increase in ACADL, the greater abundance and expression of the two subunits of isocitrate dehydrogenase tended to confirm previous observations regarding the central role of the TCA cycle in metabolic reprogramming and tumor invasiveness. Laurenti and Tennant have previously reviewed the impact of its dysregulation in cancers in association with hypoxia and increased intracellular levels of ROS [
52]. Moreover, as shown by Zeng et al., the aberrant expression of
IDH3A, which represented an upstream activator of HIF-1, promoted tumor growth and angiogenesis in various cancer types [
53].
Besides the dramatic changes observed in ACADL associated with tumor invasiveness, we also identified another protein involved in the mitochondrial translation machinery, encoded by
TUFM. This observation, which is consistent with higher abundance and expression of proteins involved in mtDNA maintenance, can be related to several interesting reports. For example, Cruz et al. found this protein in a list of five candidate biomarkers of drug-resistant ovarian cancer [
54]. Interestingly, the mitochondrial translation pathway is required for increased electron transport chain activity [
55], and its inhibition played a part in sensitizing renal cell carcinoma to chemotherapy [
56]. Chatla et al. demonstrated that TUFM was required for increased mitochondrial biosynthesis [
57]. Moreover, this latter work suggests the existence of a link with another elevated mitochondrial protein found in our study, encoded by
ALDH7A1. ALDH7A1 is an enzyme which mechanistically appeared to provide cells with protection against various forms of stress through multiple pathways [
58]. It is involved in stem cell pathways [
59,
60], and the link between its high expression and tumor invasiveness has been clearly established through the works of van den Hoogen et al. [
61] and Giacalone et al. [
62], in prostate cancer and lung cancer, respectively. Interestingly, in good agreement with our findings, Lee et al. also demonstrated its relationship with lipid catabolism as an energy source in pancreatic cancer cells [
63]. ALDH7A1 was first known as antiquitin, and the study of its subcellular localization revealed its presence in cytosol in addition to mitochondria [
64]. Finally, an intriguing feature of this enzyme, which resonated with this latter observation, was the recent work published by Babbi et al., revealing the central role played by this protein, also present in the nucleus, interacting with 23 other proteins in IntAct, and 62 in BioGRID, while
ALDH7A1 represented one of the most frequent genes in KEGG metabolic pathways [
65].
Author Contributions
Conceptualization, D.L.P., J.K. and C.R.; methodology, validation, G.O., L.R.; Preparation of samples for proteomics, D.L.P., A.B., C.H.; Preparation of samples and immunohistochemistry, S.B.; Software, validation, C.G; formal analysis, G.O., L.R.; data curation, D.L.P., J.K.; writing—original draft preparation, D.L.P., J.K.; writing—review and editing, D.L.P., J.K. and C.R.; supervision, D.L.P., J.K., C.R., C.G.; funding acquisition D.L.P., J.K. and C.R. All authors have read and agreed to the published version of the manuscript.
Figure 1.
Histological features of the four experimental rat mesothelioma tumor models. HPS staining, x400 (the scale bar represents 50 µm). Inserts (bottom right corner) represent general views (the scale bar representing 5 mm (left column) or 2.5 mm (right column)), with the open red arrows showing the location of the magnified areas.
Figure 1.
Histological features of the four experimental rat mesothelioma tumor models. HPS staining, x400 (the scale bar represents 50 µm). Inserts (bottom right corner) represent general views (the scale bar representing 5 mm (left column) or 2.5 mm (right column)), with the open red arrows showing the location of the magnified areas.
Figure 2.
Abundance changes with invasiveness, main mitochondrial proteins. A, FAO enzymes. B, ATP synthase subunits. C, Cytochrome oxidase subunits. D, TCA enzymes. Red bars represent increase and blue bars decrease, with light colors used for tendencies. Protein codes (for rattus norvegicus) are put in upper case and bold, and gene names in italics.
Figure 2.
Abundance changes with invasiveness, main mitochondrial proteins. A, FAO enzymes. B, ATP synthase subunits. C, Cytochrome oxidase subunits. D, TCA enzymes. Red bars represent increase and blue bars decrease, with light colors used for tendencies. Protein codes (for rattus norvegicus) are put in upper case and bold, and gene names in italics.
Figure 3.
Distribution of ACADL expression in rat mesothelioma tumors. A-D, Comparison of overall IHC staining with increasing invasiveness, x400 (the scale bars represent 50 µm). E, magnifications of areas of intense staining in the most aggressive, M5-T1 tumor (the scale bars represent 25 µm).
Figure 3.
Distribution of ACADL expression in rat mesothelioma tumors. A-D, Comparison of overall IHC staining with increasing invasiveness, x400 (the scale bars represent 50 µm). E, magnifications of areas of intense staining in the most aggressive, M5-T1 tumor (the scale bars represent 25 µm).
Figure 4.
Different expressions of mitochondrial genes between epithelioid and sarcomatoid MM cells. A, Mitochondrial gene expression in 10 primary MM cell lines (
Supplementary Table S3) derived from 2 different histopathological subtypes, epithelioid (EPI, n = 5) and sarcomatoid (SAR, n = 5), was analyzed with real time PCR. Data are expressed as relative mean fold increase SAR vs EPI MM cells.
B, C. MPM epithelioid (EPI UP1 and EPI UP2), and sarcomatoid (SAR UP6 and SAR UP7) cells were grown to confluence, then scratched and incubated for 24h in fresh medium (CTRL), or medium with 10 µM of etomoxir (ETOM).
B, Representative bright-field images immediately after the scratch and after 24h.
C, Cell migration. Data are presented as means ± SEM (n = 3). *
p < 0.05, ***
p < 0.001: ETOM treated cells vs CTRL cells; #
p < 0.05, ###
p < 0.001: SAR cells vs EPI cells. Scale bar is 100 µm.
Figure 4.
Different expressions of mitochondrial genes between epithelioid and sarcomatoid MM cells. A, Mitochondrial gene expression in 10 primary MM cell lines (
Supplementary Table S3) derived from 2 different histopathological subtypes, epithelioid (EPI, n = 5) and sarcomatoid (SAR, n = 5), was analyzed with real time PCR. Data are expressed as relative mean fold increase SAR vs EPI MM cells.
B, C. MPM epithelioid (EPI UP1 and EPI UP2), and sarcomatoid (SAR UP6 and SAR UP7) cells were grown to confluence, then scratched and incubated for 24h in fresh medium (CTRL), or medium with 10 µM of etomoxir (ETOM).
B, Representative bright-field images immediately after the scratch and after 24h.
C, Cell migration. Data are presented as means ± SEM (n = 3). *
p < 0.05, ***
p < 0.001: ETOM treated cells vs CTRL cells; #
p < 0.05, ###
p < 0.001: SAR cells vs EPI cells. Scale bar is 100 µm.
Figure 5.
Sarcomatoid MPM cells have higher expression of ACADL compared with epithelioid MM cells. Primary MM cells derived from 2 different histopathological subtypes, epithelioid (EPI UP1 and UP2), and sarcomatoid (SAR UP6 and UP7) were incubated in fresh medium (CTRL), or in medium with 10 µm of etomoxir (ETOM) for 24h then used for measurements. A, ACADL mRNA levels were measured with RT-PCR, in triplicate. Data are presented as means ± SEM (n = 3). ###p < 0.001: SAR cells vs EPI cells. B, ACADL protein was measured with immunoblotting in primary MM cell lines. GAPDH was used as a loading control. The figure is representative of 1 out of 3 experiments with similar results.
Figure 5.
Sarcomatoid MPM cells have higher expression of ACADL compared with epithelioid MM cells. Primary MM cells derived from 2 different histopathological subtypes, epithelioid (EPI UP1 and UP2), and sarcomatoid (SAR UP6 and UP7) were incubated in fresh medium (CTRL), or in medium with 10 µm of etomoxir (ETOM) for 24h then used for measurements. A, ACADL mRNA levels were measured with RT-PCR, in triplicate. Data are presented as means ± SEM (n = 3). ###p < 0.001: SAR cells vs EPI cells. B, ACADL protein was measured with immunoblotting in primary MM cell lines. GAPDH was used as a loading control. The figure is representative of 1 out of 3 experiments with similar results.
Figure 6.
Sarcomatoid MM cells have more active mitochondrial metabolism compared with epithelioid MM cells. Primary MM cells derived from 2 different histopathological subtypes, epithelioid (EPI UP1 and UP2), and sarcomatoid (SAR UP6 and SAR UP7), were grown in fresh medium (CTRL) or in medium with 10 µM of etomoxir for 24h then used for the following analysis. A, Fatty acid -oxidation was measured with fluorimetric assay in triplicate. Data are presented as means ± SEM (n = 3). ***p < 0.001: ETOM treated cells vs CTRL cells; #p < 0.05, ###p < 0.001: SAR cells vs EPI cells. B, The electron flux between Complex I and III was measured spectrophotometrically in triplicate. Data are expressed as means ± SEM (n = 3). *p < 0.05, ***p < 0.001: ETOM treated cells vs CTRL cells, ###p < 0.001: SAR cells vs EPI cells. C, ATP release was measured with a chemiluminescence-based assay in duplicate. Data are expressed as means ± SEM (n = 3). *p < 0.05, ***p < 0.001: ETOM treated cells vs CTRL cells; #p < 0.05, ###p < 0.001: SAR cells vs EPI cells.
Figure 6.
Sarcomatoid MM cells have more active mitochondrial metabolism compared with epithelioid MM cells. Primary MM cells derived from 2 different histopathological subtypes, epithelioid (EPI UP1 and UP2), and sarcomatoid (SAR UP6 and SAR UP7), were grown in fresh medium (CTRL) or in medium with 10 µM of etomoxir for 24h then used for the following analysis. A, Fatty acid -oxidation was measured with fluorimetric assay in triplicate. Data are presented as means ± SEM (n = 3). ***p < 0.001: ETOM treated cells vs CTRL cells; #p < 0.05, ###p < 0.001: SAR cells vs EPI cells. B, The electron flux between Complex I and III was measured spectrophotometrically in triplicate. Data are expressed as means ± SEM (n = 3). *p < 0.05, ***p < 0.001: ETOM treated cells vs CTRL cells, ###p < 0.001: SAR cells vs EPI cells. C, ATP release was measured with a chemiluminescence-based assay in duplicate. Data are expressed as means ± SEM (n = 3). *p < 0.05, ***p < 0.001: ETOM treated cells vs CTRL cells; #p < 0.05, ###p < 0.001: SAR cells vs EPI cells.
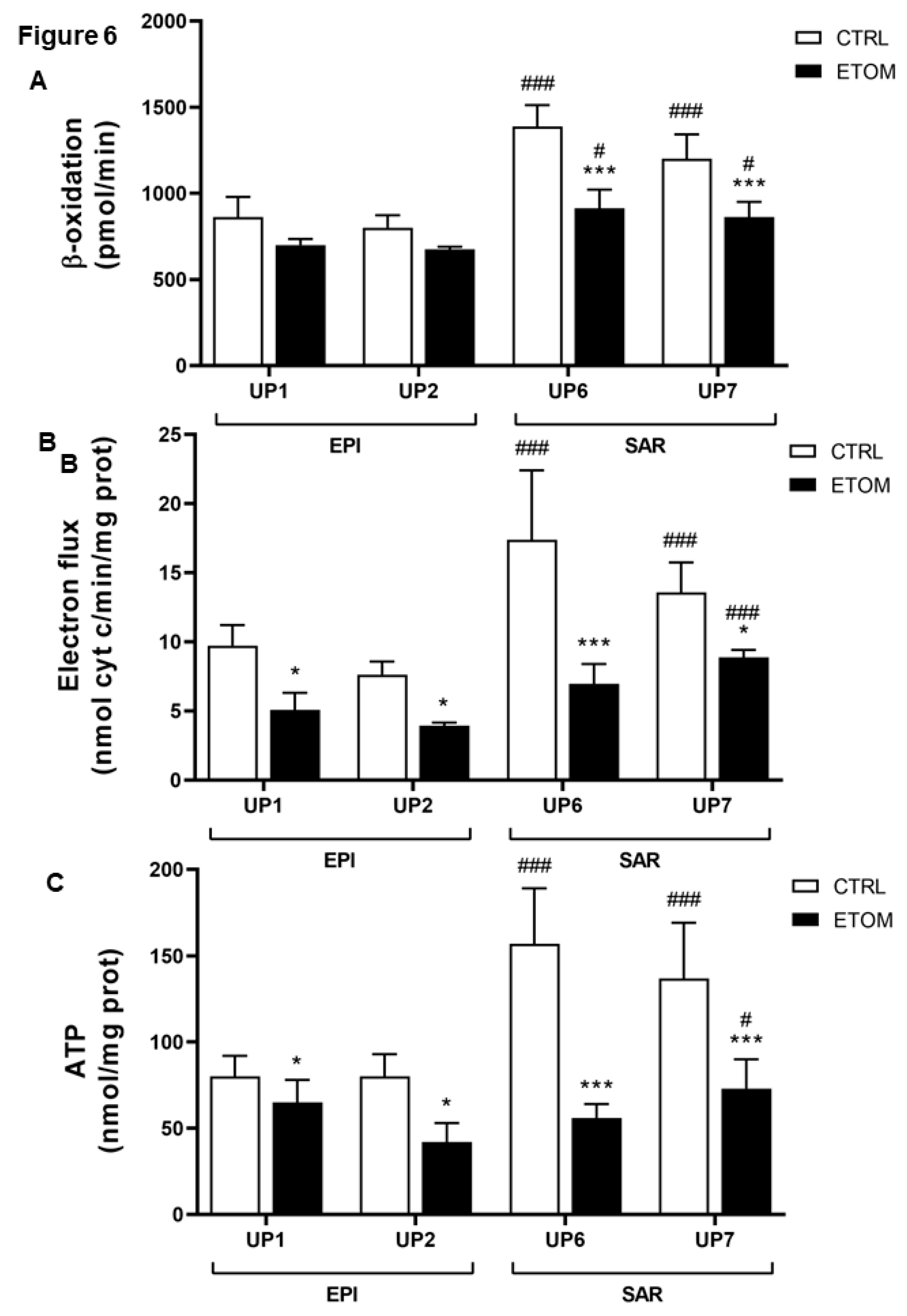
Table 1.
Origin and characteristics of human mesothelioma cell lines.
Table 1.
Origin and characteristics of human mesothelioma cell lines.
UNP |
Histotype |
Gender |
Age |
Asbestos exposure |
First line |
Second line treatment |
TTP |
OS |
1 |
Epithelioid |
M |
74 |
Unknown |
Carbo+Pem |
No |
7 |
11 |
2 |
Epithelioid |
F |
58 |
Yes |
Carbo+Pem |
Pem |
6 |
13 |
3 |
Epithelioid |
M |
76 |
Unknown |
CisPt+Pem |
No |
3 |
8 |
4 |
Epithelioid |
M |
68 |
Yes |
Carbo+Pem |
Pem |
4 |
9 |
5 |
Epithelioid |
F |
84 |
Yes |
CisPt+Pem |
No |
7 |
8 |
6 |
Sarcomatoid |
M |
80 |
Yes |
Carbo+Pem |
Trabectedin |
3 |
5 |
7 |
Sarcomatoid |
F |
78 |
Unknown |
Pem |
No |
4 |
6 |
8 |
Sarcomatoid |
M |
69 |
Yes |
Carbo+Pem |
Trabectedin |
7 |
10 |
9 |
Sarcomatoid |
F |
74 |
Unknown |
Carbo+Pem |
No |
5 |
7 |
10 |
Sarcomatoid |
M |
78 |
Yes |
Carbo+Pem |
Trabectedin |
4 |
9 |
Table 2.
Mitochondrial proteins exhibiting significant abundance changes (p < 0.05) in the three invasive rat malignant mesothelioma tumors relative to the non-invasive tumor.
Table 2.
Mitochondrial proteins exhibiting significant abundance changes (p < 0.05) in the three invasive rat malignant mesothelioma tumors relative to the non-invasive tumor.
Code #
|
Gene #
|
Full name #
|
[1 + 2 + 3] vs 4 |
ACADL |
Acadl |
Long-chain specific acyl-CoA dehydrogenase, mitochondrial |
↑ |
AL7A1* |
Aldh7a1 |
Alpha-aminoadipic semialdehyde dehydrogenase |
↑ |
ATP5H |
Atp5h |
ATP synthase subunit d, mitochondrial |
↑ |
ATPO |
Atp5o |
ATP synthase subunit O, mitochondrial |
↑ |
BCAT2* |
Bcat2 |
Branched-chain-amino-acid aminotransferase, mitochondrial |
↑ |
COX2 |
Mtco2 |
Cytochrome c oxidase subunit 2 |
↑ |
COX5B |
Cox5b |
Cytochrome c oxidase subunit 5B, mitochondrial |
↑ |
CX6C2 |
Cox6c2 |
Cytochrome c oxidase subunit 6C-2 |
↑ |
EFTU |
Tufm |
Elongation factor Tu, mitochondrial |
↑ |
HCD2 |
Hsd17b10 |
3-hydroxyacyl-CoA dehydrogenase type-2 |
↑ |
IDH3A |
Idh3a |
Isocitrate dehydrogenase [NAD] subunit alpha, mitochondrial |
↑ |
IDH3B |
Idh3b |
Isocitrate dehydrogenase [NAD] subunit beta, mitochondrial |
↑ |
KAD2 |
Ak2 |
Adenylate kinase 2, mitochondrial |
↑ |
MDHM |
Mdh2 |
Malate dehydrogenase, mitochondrial |
↑ |
MYG1* |
Myg1 |
UPF0160 protein MYG1, mitochondrial |
↑ |
OAT* |
Oat |
Ornithine aminotransferase, mitochondrial |
↑ |
PHB |
Phb |
Prohibitin |
↑ |
PHB2 |
Phb2 |
Prohibitin-2 |
↑ |
SSBP |
Ssbp1 |
Single-stranded DNA-binding protein, mitochondrial |
↑ |
TRAP1 |
Trap1 |
Heat shock protein 75 kDa, mitochondrial |
↑ |
ACADS |
Acads |
Short-chain specific acyl-CoA dehydrogenase, mitochondrial |
↓ |
ACON |
Aco2 |
Aconitate hydratase, mitochondrial |
↓ |
CISY* |
Cs |
Citrate synthase, mitochondrial |
↓ |
DECR* |
Decr1 |
2, 4 dienoyl-CoA reductase, mitochondrial |
↓ |
GSTP1* |
Gstp1 |
Glutathione S-transferase P |
↓ |
HCDH |
Hadh |
Hydroxyacyl-CoA dehydrogenase, mitochondrial |
↓ |
IVD* |
Ivd |
Isovaleryl-CoA dehydrogenase, mitochondrial |
↓ |
MGST1* |
Mgst1 |
Microsomal glutathione S-transferase 1 |
↓ |
ODO2 |
Dlst |
Dihydrolipoyllysine-residue succinyltransferase component of 2-oxoglutarate dehydrogenase complex, mitochondrial |
↓ |
PRDX3 |
Prdx3 |
Thioredoxin-dependent peroxide reductase, mitochondrial |
↓ |
RMD3* |
Rmdn3 |
Regulator of microtubule dynamics protein 3 |
↓ |
S10AA |
S100a10 |
Protein S100-A10 |
↓ |
SUOX |
Suox |
Sulfite oxidase, mitochondrial |
↓ |
THTM |
Mpst |
3-mercaptopyruvate sulfurtransferase |
↓ |
THTR |
Tst |
Thiosulfate sulfurtransferase |
↓ |
TIM9 |
Timm9 |
Mitochondrial import inner membrane translocase subunit Tim9 |
↓ |