2.1. CYP3A catalytic activity
To identify possum-specific activity differences in phase I drug metabolising enzymes, the basal catalytic activities of CYP3A in mouse, possum, avian, and human were determined (Figure 1A). The basal CYP3A catalytic activity was significantly higher (~2-fold) in mouse than in possum and avian species (p<0.05). While the basal CYP3A catalytic activities between mouse and human, and possum and avian were not significantly different (p>0.05). The mean basal CYP3A catalytic activities in these species were 0.57±0.03 (mouse), 0.35±0.01 (possum), 0.34±0.01 (avian) and 0.49±0.04 (human) in nmol/mg/min. In addition, CYP3A4 protein levels also varied between these species (Figure 1: B and C). In particular, CYP3A4 protein levels were significantly higher in possum than in avian (~4-fold). While CYP3A4 protein levels were also higher in possum, but not statistically significant (p>0.05), compared to mouse (~2-fold). The mouse CYP3A4 protein levels were ~2-fold higher than the avian. To make a comparison across target and non-target species, mouse, possum, avian and human were examined together.
Figure 1.
Basal hepatic CYP3A activity and protein levels in mouse, possum, avian and human. Liver microsomes of each species were used to determine CYP3A activity via erythromycin N-demethylation. (A). Values are expressed in nmol/mg/min. Bars represent the mean ± SEM of n=6 in triplicate (mouse and possum), n=3 in triplicate (avian) and n=2 in duplicate (pooled human liver microsomes). #Significantly different compared to possum and avian, p<0.05. The protein content for CYP3A4 in liver microsomes from 4 species was determined by Western blotting (load of protein in each well was 2 µg). Hepatic microsomes were also subjected to CYP3A4 Western blotting and the scanning densitometry results were normalised to the housekeeping protein GAPDH. (B) The bars represent the mean ± SEM of optical density of n=3 (except pooled human microsomes, n=1). (*a) Significantly different compared to avian, while (*b) Significantly different compared to mouse, p<0.05. Representative Western blot (C).
Figure 1.
Basal hepatic CYP3A activity and protein levels in mouse, possum, avian and human. Liver microsomes of each species were used to determine CYP3A activity via erythromycin N-demethylation. (A). Values are expressed in nmol/mg/min. Bars represent the mean ± SEM of n=6 in triplicate (mouse and possum), n=3 in triplicate (avian) and n=2 in duplicate (pooled human liver microsomes). #Significantly different compared to possum and avian, p<0.05. The protein content for CYP3A4 in liver microsomes from 4 species was determined by Western blotting (load of protein in each well was 2 µg). Hepatic microsomes were also subjected to CYP3A4 Western blotting and the scanning densitometry results were normalised to the housekeeping protein GAPDH. (B) The bars represent the mean ± SEM of optical density of n=3 (except pooled human microsomes, n=1). (*a) Significantly different compared to avian, while (*b) Significantly different compared to mouse, p<0.05. Representative Western blot (C).
Since differences in the basal catalytic activities of CYP3A were found in the test species, a compound library on the structural features of known CYP450 inhibitors was then examined for possum-specific differences in inhibition. These 59 compounds were initially screened with estimated IC50 and 2-fold IC50 values (Supplementary Table 1). Compounds with previously unreported IC50 values were screened at concentrations of 1 and 5 μM. From this initial screen, 24 compounds that showed the greatest inhibition against possum CYP3A were selected for further examination. Of these 24 compounds, none showed significant inhibition (<50% of control) of CYP3A enzyme activity in possum liver microsomes at estimated IC50 and 2-fold IC50 values or at concentrations of 1 and 5 μM (Supplementary Table 2). However, 12 compounds reduced the catalytic activity of possum CYP3A to some extent, mainly at a concentration of 5 μM or estimated 2-fold IC50 values, but these reduced catalytic activities were not significantly different from their respective controls (Figure 2A). These compounds were WAY-325945 (65%), ketoconazole (73%), cobicistat (76%), terfenadine (79%), benzbromarone (80%), WAY-657644 (81%), ritonavir (81%), gentiopicroside (82%), WAY-325412 (83%), chlorzoxazone (83%), galeterone (83%), and alizarin (84%) of the control. In order to determine species-specific differences, these compounds were then examined in the mouse. Interestingly, ketoconazole was the only compound that significantly reduced mouse CYP3A catalytic activity at IC50 and 2-fold IC50 values (40-46% of control, p<0.05) (Figure 2B). While other compounds such as WAY-325945 (67%), cobicistat (68%), 2’-hydroxyacetophenone (75%), terfenadine (83%), ritonavir (84%) and WAY-657644 (88%) showed some level of inhibition in mouse liver microsomes at a concentration of 5 μM or an estimated 2-fold IC50 value. However, these compounds were not statistically significant from their respective controls. A further six compounds compared from the list of 12 compounds that showed some reduction in possum CYP3A catalytic activities were further examined at a higher concentration (10 μM) in possum and mouse liver microsomes. This was done to determine if the tested compounds would further decrease the catalytic activities of CYP3A, particularly in possums. The results showed that these 6 compounds (alizarin, galeterone, cobicistat, gentiopicroside, chlorzoxazone, and benzbromarone) did not show any significant inhibition of CYP3A catalytic activities in either species at 10 μM (Figure 3).
Figure 2.
Effect of CYP450 inhibitor-based compounds on CYP3A catalytic activity in possum and mouse liver microsomes. Possum (A) and mouse (B) liver microsomes were incubated with 0.1 M phosphate buffer and CYP450 inhibitor-based compounds, and erythromycin N-demethylation was determined as an indicator of CYP3A inhibitory catalytic activity at estimated IC50 and 2-fold IC50 values. The dotted line shows the 50% control of CYP3A enzymes. The bars represent the mean ± SEM of n=3 in duplicate in percent control. *Significantly different from the respective solvent control. Abbreviated compound names refer to WAY-325945 (WAY-3259), ketoconazole (Keto), cobicistat (Cobi), terfenadine (Terf), benzbromarone (Benz), WAY-657644 (WAY-6576), ritonavir (Rito), gentiopicroside (Genti), WAY-325412 (WAY-3254), chlorzoxazone (Chlor), galeterone (Gale), and alizarin (Ali).
Figure 2.
Effect of CYP450 inhibitor-based compounds on CYP3A catalytic activity in possum and mouse liver microsomes. Possum (A) and mouse (B) liver microsomes were incubated with 0.1 M phosphate buffer and CYP450 inhibitor-based compounds, and erythromycin N-demethylation was determined as an indicator of CYP3A inhibitory catalytic activity at estimated IC50 and 2-fold IC50 values. The dotted line shows the 50% control of CYP3A enzymes. The bars represent the mean ± SEM of n=3 in duplicate in percent control. *Significantly different from the respective solvent control. Abbreviated compound names refer to WAY-325945 (WAY-3259), ketoconazole (Keto), cobicistat (Cobi), terfenadine (Terf), benzbromarone (Benz), WAY-657644 (WAY-6576), ritonavir (Rito), gentiopicroside (Genti), WAY-325412 (WAY-3254), chlorzoxazone (Chlor), galeterone (Gale), and alizarin (Ali).
Figure 3.
Effect of CYP450 inhibitor-based compounds on CYP3A catalytic activity in mouse and possum liver microsomes. Possum and mouse liver microsomes were incubated with 0.1 M phosphate buffer and CYP450 inhibitor-based compounds, and erythromycin N-demethylation was determined at a higher concentration (10 µM) as an indicator of CYP3A inhibitory catalytic activity. The bars represent the mean ± SEM of n=3 in duplicate. Data were analysed using one-way ANOVA and none were statistically different compared to controls. Abbreviated compound names refer to alizarin (Ali), galeterone (Gale), cobicistat (Cobi), gentiopicroside (Genti), chlorzoxazone (Chlor), and benzbromarone (Benz).
Figure 3.
Effect of CYP450 inhibitor-based compounds on CYP3A catalytic activity in mouse and possum liver microsomes. Possum and mouse liver microsomes were incubated with 0.1 M phosphate buffer and CYP450 inhibitor-based compounds, and erythromycin N-demethylation was determined at a higher concentration (10 µM) as an indicator of CYP3A inhibitory catalytic activity. The bars represent the mean ± SEM of n=3 in duplicate. Data were analysed using one-way ANOVA and none were statistically different compared to controls. Abbreviated compound names refer to alizarin (Ali), galeterone (Gale), cobicistat (Cobi), gentiopicroside (Genti), chlorzoxazone (Chlor), and benzbromarone (Benz).
In addition, 19-21 compounds that showed potential differences in CYP3A inhibitory activity in possum and mouse were further examined in avian and human liver microsomes. None of the test compounds showed any significant inhibition (<50% of control) of catalytic activity in either species. Nonetheless, in avian liver microsomes, most of the compounds showed some level of inhibition at 5 μM concentrations or the estimated 2-fold IC50 values, but these did not differ significantly from their respective controls.
2.1. P-nitrophenol glucuronidation activity
To identify possum-specific differences in phase II drug metabolising enzymes, basal UGT2B glucuronidation activity was determined. Possum had significantly higher p-nitrophenol glucuronidation activity than the avian (~8-fold) and mouse (~1.5-fold) (Figure 4A). The mouse also had ~5-fold higher p-nitrophenol glucuronidation activity than the avian. The basal p-nitrophenol glucuronidation activities in these species were 3.60±0.17 (possum), 3.44±0.13 (human), mouse (2.44±0.18) and avian (0.46±0.04) in μmol/mg/min. Additionally, UGT2B4 protein levels also varied between species (Figure 4B and 4C). The UGT2B4 protein levels from pooled human liver microsomes were higher than those from avian (~3-fold), possum (~3-fold), and mouse (~2-fold). Furthermore, the mouse hepatic UGT2B4 protein levels were significantly increased from the possum and mouse.
Figure 4.
P-nitrophenol glucuronidation activity in mouse, possum, avian and human liver microsomes. Liver microsomes of each species were incubated with 0.1 M phosphate buffer and <10% DMSO, and the p-nitrophenol glucuronidation activity was determined as an indicator of UGT glucuronidation activity (A). Values are expressed as µmol/mg/min. Bars represent the mean ± SEM of n=6 in triplicate for possum and mouse, n=3 in triplicate for avian, and n=2 in duplicate for pooled human liver microsomes. (*a) Significantly different from avian and (*b) Significantly different from mouse, p<0.05. The protein content for UGT2B4 in hepatic microsomes from 4 species was determined by Western blotting (load of protein in each well was 2 µg). UGT2B4 Western blots (B) and scanning densitometry results normalized to the housekeeping protein GAPDH (C). The bars represent the mean ± SEM of optical density from n=3 (except for pooled human liver microsomes, n=1). *Significantly different compared to solvent control, p<0.05.
Figure 4.
P-nitrophenol glucuronidation activity in mouse, possum, avian and human liver microsomes. Liver microsomes of each species were incubated with 0.1 M phosphate buffer and <10% DMSO, and the p-nitrophenol glucuronidation activity was determined as an indicator of UGT glucuronidation activity (A). Values are expressed as µmol/mg/min. Bars represent the mean ± SEM of n=6 in triplicate for possum and mouse, n=3 in triplicate for avian, and n=2 in duplicate for pooled human liver microsomes. (*a) Significantly different from avian and (*b) Significantly different from mouse, p<0.05. The protein content for UGT2B4 in hepatic microsomes from 4 species was determined by Western blotting (load of protein in each well was 2 µg). UGT2B4 Western blots (B) and scanning densitometry results normalized to the housekeeping protein GAPDH (C). The bars represent the mean ± SEM of optical density from n=3 (except for pooled human liver microsomes, n=1). *Significantly different compared to solvent control, p<0.05.
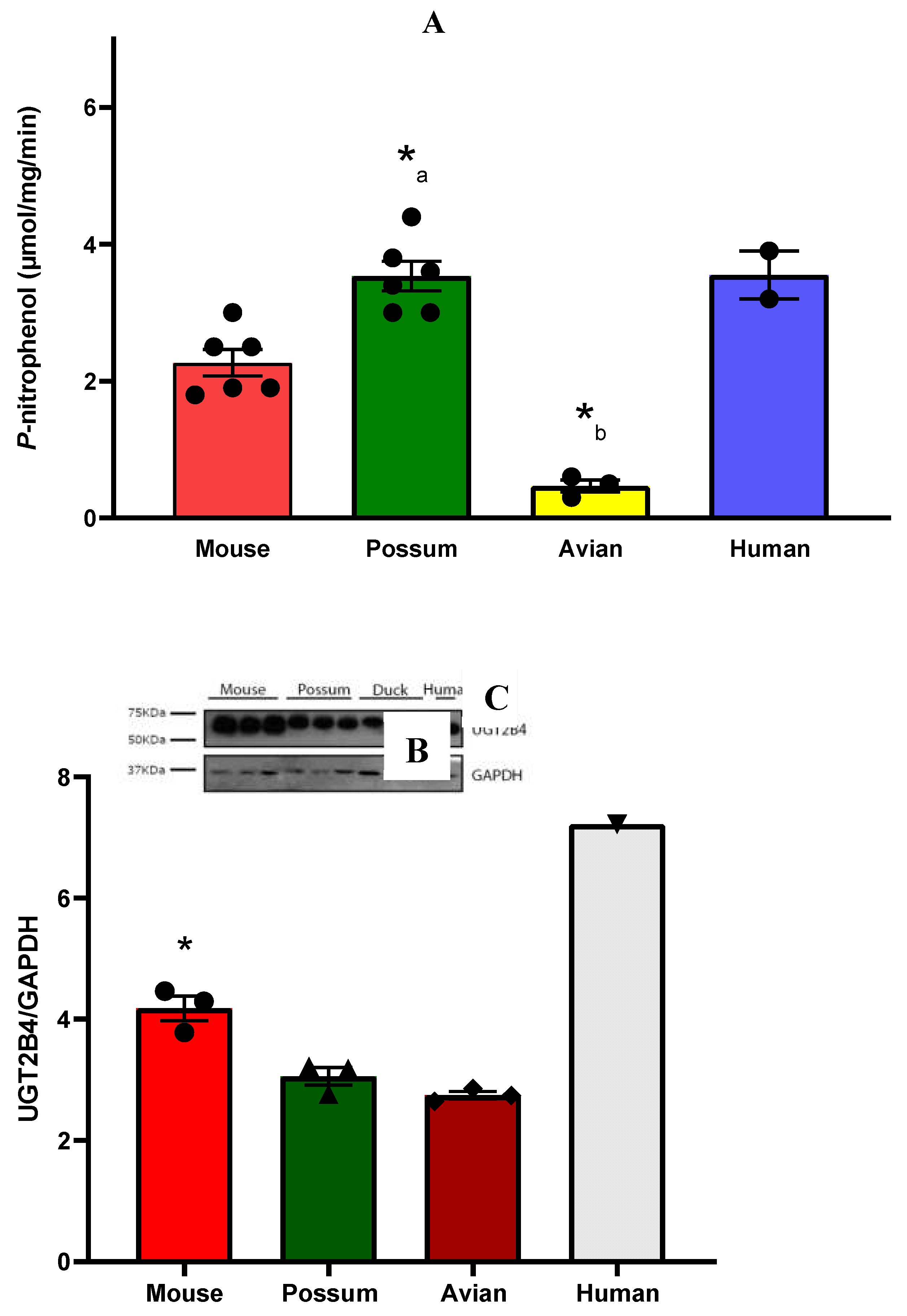
To examine the possum-specific differences in UGT enzymes, 17 compounds were further screened for their potential to inhibit UGT2B enzymes in possum, mouse, avian, and human liver microsomes (Supplementary Table 3). Compounds such as isosilybin (65%), ketoconazole (72%) and fluconazole (74%) showed reduced UGT2B glucuronidation activity in possums, mainly at 2-fold IC50 values compared to their respective controls (p<0.05). Even at 2-fold IC50 values, these reduced UGT2B inhibitory activities were not less than 50% of control (Figure 5). When one of these compounds (isosilybin) was further tested for its inhibitory potential on glucuronidation activity with varying concentrations of p-nitrophenol (substrate), it also showed no significant changes compared to their respective control activities (Table 1). Similarly, in the mouse, compounds such as fluconazole (78%) and cyclosporin A (80%) reduced p-nitrophenol glucuronidation activity at 2-fold IC50 values compared to their respective controls (p<0.05), but these inhibitory activities did not fall below 50%. In addition, activity elicited by most of the test compounds in human liver microsomes was over 88% of control. Even at 2-fold IC50 values, there was no major inhibition. Specifically, the compounds screened in human liver microsomes were itraconazole (88%), cobicistat (90%) and fluconazole (94%). In addition, most of the compounds examined showed reduced p-nitrophenol glucuronidation activity (ranging from 33-87% of control) in avian species, but these did not differ significantly from their respective controls.
Figure 5.
Compound-mediated inhibition of p-nitrophenol glucuronidation activity in possum and mouse liver microsomes. Liver microsomes of possum (A) and mouse (B) were incubated with 0.1 M phosphate buffer and inhibitory compounds, and p-nitrophenol glucuronidation activity was determined as an indicator of UGT glucuronidation activity. * Significantly different from solvent control, p<0.05. The bars represent the mean ± SEM of n=3 in duplicate in percent control. Abbreviated compound names refer to isosilybin (Isosi), ketoconazole (Keto), fluconazole (Fluco), and cyclosporin A (Cyclo).
Figure 5.
Compound-mediated inhibition of p-nitrophenol glucuronidation activity in possum and mouse liver microsomes. Liver microsomes of possum (A) and mouse (B) were incubated with 0.1 M phosphate buffer and inhibitory compounds, and p-nitrophenol glucuronidation activity was determined as an indicator of UGT glucuronidation activity. * Significantly different from solvent control, p<0.05. The bars represent the mean ± SEM of n=3 in duplicate in percent control. Abbreviated compound names refer to isosilybin (Isosi), ketoconazole (Keto), fluconazole (Fluco), and cyclosporin A (Cyclo).
Table 1.
Substrate-dependent effect of isosilybin on p-nitrophenol glucuronidation activity in possum liver microsomes.
Table 1.
Substrate-dependent effect of isosilybin on p-nitrophenol glucuronidation activity in possum liver microsomes.
Compound |
p-nitrophenol concentration |
Concentration (µM) |
Glucuronidation activities+
|
Percent of control |
Isosilybin |
2.5 mM |
0 |
0.65±0.15 |
100 |
100 |
0.47±0.14 |
75 |
200 |
0.72±0.32 |
109 |
|
|
|
|
|
Isosilybin |
1.25 mM |
0 |
0.30±0.07 |
100 |
100 |
0.15±0.01 |
56 |
200 |
0.17±0.02 |
70 |
|
|
|
|
|
Isosilybin |
0.625 mM |
0 |
0.18±0.02 |
100 |
100 |
0.12±0.09 |
63 |
200 |
0.13±0.05 |
67 |